Introduction
Quantum entanglement is a foundational resource for quantum computing, communication, and sensing. Across different physical platforms, researchers have realized entangled states in the laboratory, pushing toward practical quantum technologies. This report examines experimental implementations of entanglement in five major platforms: photonic systems (entangled photons), trapped-ion qubits, superconducting qubits, quantum sensing setups, and semiconductor quantum dots. For each platform, we outline the key hardware components, discuss practical challenges and limitations, and highlight recent advancements and emerging trends in entanglement-based applications.
Entangled Photons in Photonic Systems
Photonic entanglement typically involves pairs of photons whose quantum states (such as polarization, energy-time, or momentum) are interlinked. Photons are ideal carriers of entanglement for quantum communication due to their speed and low interaction with the environment. Experimentally, photonic entanglement has been realized both in bulk optical setups and on integrated photonic chips.
Key Hardware Components:
- Entangled Photon Sources: Nonlinear optical crystals (e.g., BBO, PPKTP) or waveguides for spontaneous parametric down-conversion (SPDC) and four-wave mixing are used to generate entangled photon pairs. For example, a high-brightness source was demonstrated using type-0 phase-matched SPDC in a Sagnac interferometer loop [1], [2].
- Pump Lasers: A stable laser (often ultraviolet or visible) pumps the nonlinear medium to produce photon pairs. High-power continuous-wave lasers (hundreds of mW) have been used to boost pair production without damaging the crystal [1].
- Photonic Circuitry: Beam splitters, phase shifters, polarizers, and fibers or integrated waveguides guide and manipulate entangled photons. In integrated photonics, on-chip resonators and interferometers can create and process entangled states.
- Detectors and Readout: Single-photon detectors (avalanche photodiodes or superconducting nanowire detectors) are required to detect individual photons and perform coincidence measurements. A complete entanglement distribution system includes a source, a transmission channel (fiber or free-space link), and photon detectors [1].
Practical Challenges and Limitations:
- Loss and Collection Efficiency: Photons can be lost in transmission or during collection into fibers, reducing entanglement distribution rates. High end-to-end efficiency (from source to detection) is critical – experiments have achieved up to ~84% collection efficiency to close detection loopholes in Bell tests. Loss limits the distance over which entanglement can be shared without quantum repeaters [1].
- Entanglement Generation Rate: SPDC sources generate photon pairs probabilistically. Pushing to high pair rates is challenging because increasing pump power also raises multi-pair emissions that degrade entanglement fidelity (due to the Poissonian nature of SPDC). Techniques like wavelength-division multiplexing have been used to scale up entanglement generation by running many SPDC processes in parallel[1].
- Multi-Photon Entanglement Scaling: Creating entanglement among more than two photons (for example, four-photon GHZ states or higher) becomes exponentially difficult. Probabilistic sources and detector inefficiencies mean that experiments with 8 or 10 photon entanglement require enormous trial numbers and suffer low success rates.
- Stability and Interference: Entangled photons are sensitive to optical path length fluctuations. Maintaining phase stability in interferometers (especially for time-bin or energy-time entanglement) is non-trivial, particularly outside the lab environment.
Recent Advancements and Emerging Trends:
- High-Quality Entangled Sources: New SPDC source designs have dramatically improved performance. A recent source achieved simultaneous high pair rate, broad bandwidth, low loss, and 99%+ state fidelity. Such sources enabled entanglement-based quantum key distribution (QKD) at record rates, with simulations indicating the potential for >1 Gbps secure key rates. This is a leap from earlier demonstrations (e.g., a satellite-to-ground entanglement experiment achieved only ~5.9 MHz pair rate and a few bits per second of key rate) [1].
- Integrated Photonic Entanglement: Progress in silicon photonics and other integrated platforms allows on-chip generation and processing of entangled photons. Researchers have demonstrated entangled photon-pair sources in silicon nitride, lithium niobate, and silicon chips, covering telecom wavelengths for compatibility with fiber networks. Integration promises improved stability and scalability for complex photonic entangled-state circuits (e.g. for photonic quantum computing or communication nodes) [2].
- Long-Distance Entanglement Distribution: Field experiments have distributed polarization-entangled photons over tens of kilometers of optical fiber and even between ground stations and satellites. For instance, entangled photons from a quantum dot source were used for QKD across two buildings connected by 350 m of fiber. Free-space links have extended entanglement to satellite scales, laying groundwork for a future “quantum internet” [3]
- Multiphoton and High-Dimensional Entanglement: Beyond simple two-photon entanglement, experiments are exploring hyper-entanglement (entanglement in multiple degrees of freedom) and high-dimensional entangled states (using more than two levels per photon). These exotic states can carry more information and have been shown in laboratory conditions (e.g., entangling photons in polarization and orbital angular momentum). While mostly exploratory, they point to richer forms of photonic entanglement for sensing and communication.
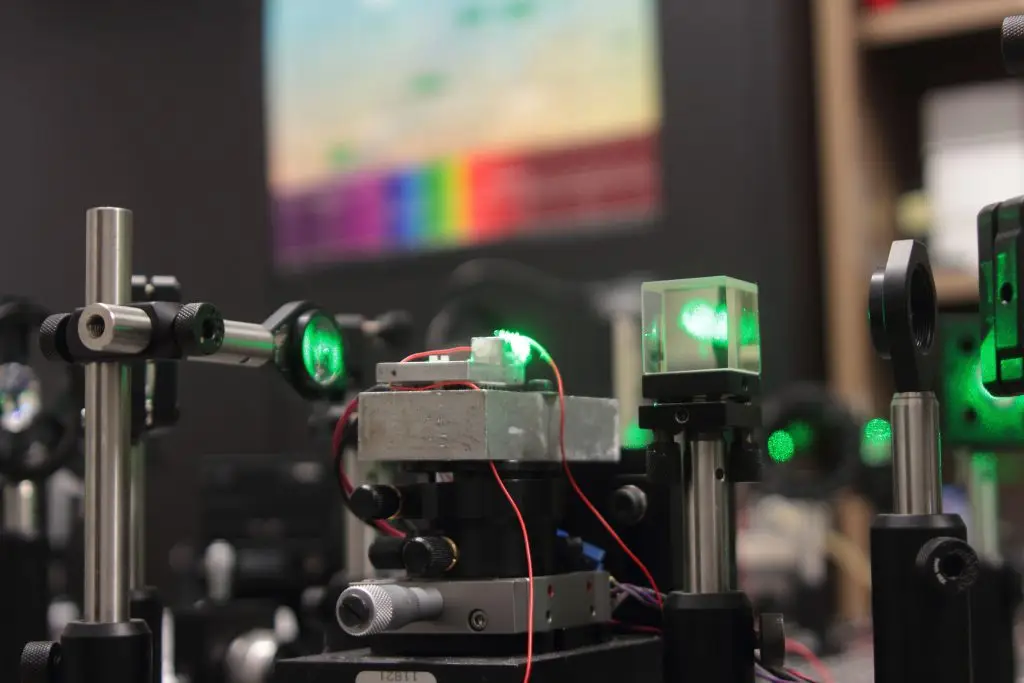
Entanglement in Trapped-Ion Systems
Trapped ions are one of the most mature platforms for quantum computing, known for excellent qubit coherence and high-fidelity gate operations. Entanglement is routinely generated between ions using laser-mediated interactions. In fact, some of the largest experimentally entangled states (by number of particles) have been realized with trapped-ion qubits. A linear chain of ions can be entangled in GHZ states or used to implement quantum logic operations for algorithms.
Key Hardware Components:
- Ion Trap: A radio-frequency (RF) Paul trap or Penning trap confines ions (such as Yb⁺, Ba⁺, Ca⁺) in free space using electric/magnetic fields. Modern traps are micro-fabricated with segmented electrodes to allow shuttling ions and scaling to many trapping zones. Vacuum systems (often ultra-high vacuum) are essential to isolate ions from air collisions.
- Laser Systems: Lasers perform multiple roles – cooling the ions to near the motional ground state, initializing and reading out qubit states (via optical pumping and fluorescence detection), and driving entangling gates. For example, in a common two-qubit entangling gate (Mølmer–Sørensen gate), a pair of ions is illuminated with bichromatic laser beams that excite a shared vibrational mode, producing an effective spin–spin coupling and entanglement. Precise laser frequency, phase, and pulse shaping control are required.
- Control and Detection: Each ion is typically individually addressed by tightly focused laser beams or acousto-optic deflectors. Photo-multiplier tubes or EMCCD cameras collect fluorescence from ions to read out their states (bright = |1>, dark = |0>, for instance). The hardware also includes stable RF sources for the trap, magnetic field coils for splitting energy levels, and sometimes microwave sources if hyperfine qubits are driven by microwaves.
- Auxiliary Systems: To scale up, ion experiments use components like high numerical aperture imaging optics (to detect and perhaps even couple photons from ions for remote entanglement), and sometimes multiple trap modules interconnected by photonic links (for ion-photon entanglement interfaces).
Practical Challenges and Limitations:
- Scalability and Loading: While a few ions are easy to trap and entangle, handling dozens of ions in one trap is challenging. In a single chain, too many ions lead to closely spaced vibrational modes and spectral crowding, making it hard to perform selective entangling gates. The largest entangled ion state to date is 32 ions in a GHZ state, but scaling beyond that in one zone requires innovations (the control complexity and mode heating increase with chain length). Loading large numbers of ions and reordering or separating them for specific gates (the Quantum CCD concept) adds operational overhead[4].
- Gate Speed vs. Fidelity: Ion gates, performed with lasers, are relatively slow (typically tens of microseconds, slower than solid-state qubit gates by orders of magnitude). Faster gates would induce unwanted motion or off-resonant excitations. While two-qubit gate fidelities can be very high (~99.9% in small systems), performing many sequential gates is time-consuming and could allow more decoherence. Competing platforms like superconductors operate faster, but ions trade speed for fidelity and all-to-all connectivity[5].
- Control Complexity: Each ion requires precise laser alignment and calibration. Cross-talk between neighboring ion qubits can occur if lasers are misaligned. Additionally, the requirement of multiple laser wavelengths (for cooling, repumping, gates, etc.) and optical stability makes the system complex. Increasing qubit count demands an exponential increase in parallel laser beams or more sophisticated beam delivery (like multichannel acousto-optic modulators), which is experimentally demanding.
- Environmental Disturbances: Though well-isolated, ions are still sensitive to electric-field noise (which can cause motional heating) and magnetic field fluctuations (causing qubit phase errors). Maintaining long entanglement coherence length (the time the entangled state remains intact) requires mitigation of these noise sources, e.g., using magnetic shielding and improved trap electrode surfaces to reduce charging and noise.
Recent Advancements and Trends:
- Larger Entangled States: Ion trap quantum computers have steadily grown in qubit number. In 2023, a trapped-ion system successfully created a 32-ion GHZ entangled state with high fidelity. This is a landmark for controlled entanglement size in any platform, only recently surpassed by superconducting circuits (60 qubits) in 2024. Multi-ion entanglement is also used in quantum simulations and error-correcting codes on ion processors[4].
- Modular and Scalable Architectures: To overcome scaling limits of a single trap, researchers are developing modular ion trap architectures. One approach is the quantum charge-coupled device (QCCD) concept, where ions are shuttled between different trap zones for interacting only when needed. A “race-track” trap design demonstrated integration of technologies like electrode broadcasting and fast ion transport while maintaining high gate fidelities. Another approach is networking multiple ion traps: recent experiments entangled ions in separate traps connected by photonic links, including a 2023 demonstration of entangling ions over 230 m of optical fiber. Such efforts pave the way for distributed ion-based quantum networks[4] [6].
- High-Fidelity Gates and Error Correction: Continuous improvements in laser stability and trap design have pushed two-qubit gate fidelities above 99%. With these gains, small-scale demonstrations of quantum error correction have been carried out on trapped-ion systems. These experiments entangle multiple ions to create logical qubits that can detect and correct errors, an essential step toward fault-tolerant quantum computing[4].
- Hybrid Entanglement Schemes: Trapped ions are also used in hybrid systems; for example, entangling an ion’s state with a photon for quantum networking. Remote ion-ion entanglement via photons has been shown, and is an area of active research for quantum repeaters. Additionally, dual-species ion chains (one species for qubits, another for cooling) improve performance by allowing continuous cooling without disturbing quantum information – indirectly helping sustain entanglement in larger systems.
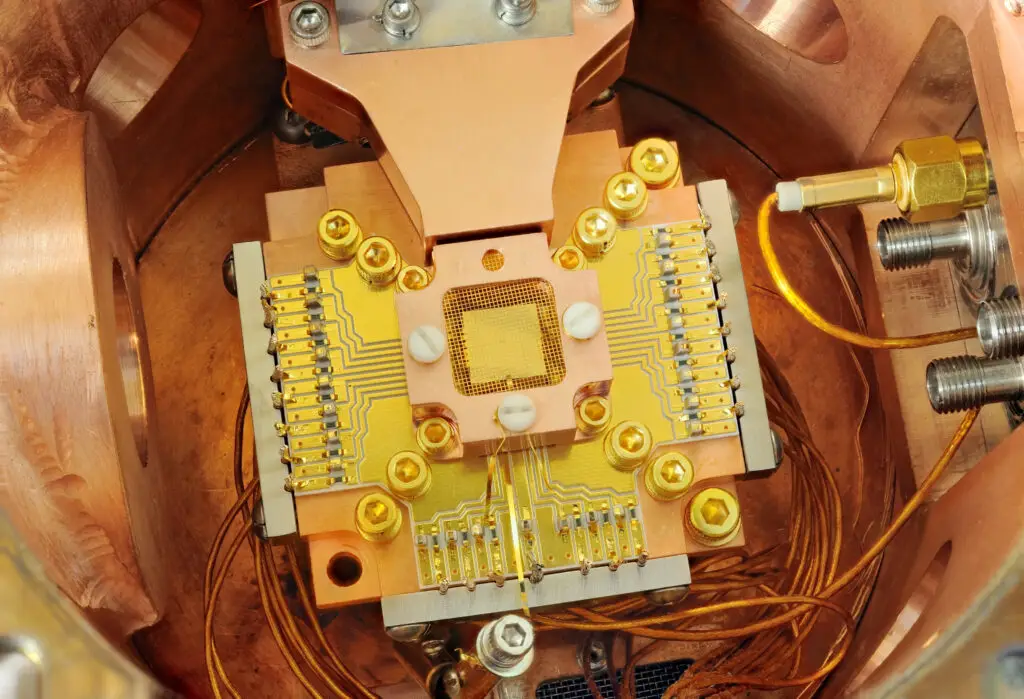
NIST physicists used this apparatus to coax two beryllium ions (electrically charged atoms) into swapping the smallest measurable units of energy back and forth, a technique that may simplify information processing in a quantum computer. The ions are trapped about 40 micrometers apart above the square gold chip in the center. The chip is surrounded by a copper enclosure and gold wire mesh to prevent buildup of static charge. Credit: Y. Colombe/NIST Disclaimer: Any mention of commercial products within NIST web pages is for information only; it does not imply recommendation or endorsement by NIST.
Superconducting qubits (such as the transmon design) are electric circuits that behave as artificial atoms, operating at millikelvin temperatures. They are a leading platform for quantum computing due to fast gate speeds and integrability. Entanglement between superconducting qubits is generated via microwave-frequency interactions on-chip, and such entangled states underpin algorithms and error correction codes. Recent experiments have dramatically increased the number of superconducting qubits that can be entangled simultaneously.
Key Hardware Components:
- Superconducting Qubits: Typically Josephson junction-based circuits (e.g., transmons) that act as non-linear oscillators with two lowest levels forming a qubit. These qubits are fabricated on a chip (using aluminum or niobium superconducting layers). They are placed in 2D arrays or 1D chains with fixed or tunable couplers between qubits to mediate entangling interactions.
- Cryogenic Environment: A dilution refrigerator cools the qubits to ~10–20 millikelvin, well below the superconducting critical temperature, to eliminate thermal excitations. The cryostat also provides shielding from external electromagnetic noise.
- Microwave Control and Readout: Each qubit has control lines for delivering microwave pulses that drive rotations and entangling gates. Entanglement is often achieved through two-qubit gates like controlled-NOT or iSWAP, implemented by coupling qubits via a microwave resonator bus or a tunable coupler. Readout resonators coupled to each qubit allow measurement via microwave reflections – to observe entanglement, one typically performs correlated measurements on multiple qubits. High-speed electronics (AWGs, FPGA controllers) orchestrate sequences with nanosecond timing.
- Amplifiers and Filtering: Superconducting quantum circuits require ultra-low-noise amplification for readout signals (e.g., Josephson parametric amplifiers at the millikelvin stage) and extensive filtering to avoid stray noise entering the system. These are crucial for preserving entangled states long enough to measure and use them.
Practical Challenges and Limitations:
- Coherence Time: Superconducting qubits have finite coherence (due to interactions with two-level system defects, radiation loss, etc.). Typical coherence times are on the order of 50–300 microseconds for state-of-the-art transmons. This limits how long large entangled states can survive. A GHZ state of many qubits will start decohering one qubit at a time, quickly reducing its fidelity. The recent 60-qubit GHZ state, for example, was transient and required fast creation and measurement before decoherence set in[7].
- Gate Fidelity vs Qubit Count: Entangling two superconducting qubits with ~99% fidelity is routine, but maintaining high fidelity across many qubits is harder. Calibration becomes complex as qubit numbers grow. Moreover, limited connectivity means entangling distant qubits requires intermediate swaps, compounding errors. Errors scale up with qubit count, so entangling, say, 60 qubits in a GHZ state as one collective operation is at the edge of current capabilities and required a special protocol to manage errors[7].
- Cross-Talk and Control Complexity: With dozens of control lines in a small chip, microwave cross-talk between qubits can inadvertently entangle or mix states. Isolation and careful frequency planning (to avoid spectral collisions between qubits and crosstalk between control channels) are needed. The control hardware overhead (DACs, cables, etc.) also grows with qubit number, making it challenging to scale physically beyond a few hundred qubits without new techniques (like multiplexing control lines or using cryo-CMOS controllers).
- Fabrication Variability: Superconducting circuits can suffer from device-to-device variability (e.g., variation in resonant frequencies, coupler strength). This can affect the uniformity of entangling operations. In large entangled states (like multi-qubit GHZ), slight frequency detunings can cause phase errors. Advanced fabrication and in-situ tuning elements (like magnetic flux bias for each qubit/coupler) are used to mitigate this, but at the cost of additional complexity.
Recent Advancements and Trends:
- Record-Size Entangled States: As of early 2024, the largest reported genuine entangled state in any platform is a 60-qubit GHZ state on a superconducting chip. Researchers at Zhejiang University entangled 60 transmon qubits in a 2D lattice, nearly doubling the previous record (32 ions). This experiment used a scalable protocol to create global entanglement and even explored techniques to protect the GHZ state from decoherence using periodic driving (a form of dynamical error suppression). Such demonstrations show that superconducting platforms can achieve massive entangled states, important for quantum computational power and simulation of many-body physics[7][8].
- Error Correction and Entanglement: Superconducting qubits have demonstrated entanglement in service of quantum error correction. For example, entangling ~17 qubits into a surface-code logical qubit or generating multipartite entanglement for a repetition code has been achieved on devices from IBM and Google. In 2023, Google reported entangling 49 qubits in a cluster state as part of an error-corrected logical qubit demonstration (though with fidelity below threshold for full fault tolerance). These are key steps toward using entanglement to detect/correct errors in real time.
- Improved Coherence and Materials: There is a strong trend of materials research and engineering advances that extend coherence, which directly benefits entanglement. New fabrication techniques yield junctions and substrates with fewer two-level defects, and 3D integration reduces loss. Longer coherence means deeper circuits and the ability to maintain entangled states longer. Concurrently, fast high-fidelity two-qubit gates (90–99.5% in <100 ns) are being developed to create entanglement more quickly relative to coherence time, improving the net entangled-state fidelity.
- Quantum Networks and Entanglement Distribution: While most superconducting qubit entanglement is on a single chip, initial steps to entangle qubits between chips are underway. Researchers have used microwave transmission lines to entangle qubits across a 1-meter distance (achieving ~94% fidelity Bell pairs after entanglement purification). Efforts to link superconducting processors via optical photons (using microwave-to-optical transducers) are also in progress. These developments hint at distributed superconducting quantum computing, where entanglement is shared between modules to scale beyond the confines of one cryostat.
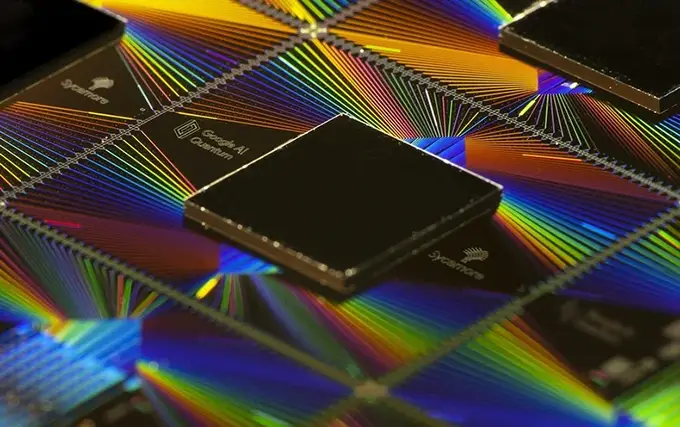
Google’s superconducting Sycamore chip which demonstrated “quantum supremacy”
Entanglement-Enhanced Quantum Sensing
Quantum sensing involves exploiting quantum states (including entanglement) to achieve measurement precision beyond classical limits. Entanglement can non-trivially correlate the particles or fields used as probes, enabling, for example, improved signal-to-noise or resolution. Unlike the other sections (which focus on a hardware platform), here we focus on how entanglement is implemented in various experimental sensors – from atomic clocks to magnetometers and optical interferometers – and what practical benefits and challenges arise.
Key Use Cases and Hardware:
- Optical Atomic Clocks: Entanglement is used to surpass the Standard Quantum Limit (SQL) set by uncorrelated atoms in precision frequency measurements. State-of-the-art optical lattice clocks interrogate thousands of atoms; by preparing the atoms in an entangled (spin-squeezed) state, one can reduce quantum projection noise. Hardware includes ultra-stable lasers and optical cavities that mediate entangling interactions between atoms. For example, a cavity QED setup can entangle an ensemble of Sr or Yb atoms by measurement-induced spin squeezing, effectively linking their quantum phase fluctuations. Atomic clocks with entangled atoms have been shown to operate below the classical noise floor, directly demonstrating improved stability at the 10−17 level[9].
- Magnetometers and Atomic Sensors: Ensembles of cold atoms or NV centers in diamond can be entangled to improve sensitivity to magnetic fields. In practice, spin-squeezing (a form of multi-particle entanglement) is generated via interactions (for cold atoms, often via collisions or cavity feedback). Hardware like high-finesse optical cavities or optical lattices create the requisite entangling interactions among atomic spins. Recently, entangled states of ~10,000 atoms were used in magnetometry to beat the SQL, achieving several dB of noise reduction beyond what independent atoms could do.
- Optical Interferometry (NOON States): In photonic sensing, entangled photon states such as NOON states (e.g., two photons in superposition of both going down two paths, or more generally N photons) can achieve phase supersensitivity. Experimental realizations include 2-photon and 4-photon NOON states used in interferometers to measure phase shifts with enhanced resolution (N photons can in principle give N-fold phase precision). Hardware-wise, this involves multi-photon entangled sources (often SPDC producing two pairs and then interfering them to create a 4-photon entangled state) and stable interferometric setups. Due to losses, demonstrations have been limited to small N, but they prove the concept of entanglement-enhanced interferometry.
- Quantum Illumination & Radar: Entangled or correlated photon beams can improve detection of objects in noisy environments. Experiments in microwave quantum illumination have used entangled microwave photons (generated by Josephson parametric amplifiers) to detect low-reflectivity targets with gains over classical states. This hardware is specialized but shows the versatility of entanglement in sensing beyond optical frequencies.
Practical Challenges:
- Decoherence vs. Sensing Gain: Entanglement is fragile – any decoherence can destroy the correlations that give the sensing advantage. In a sensor, the very act of sensing often requires the system to interact with the environment (e.g., atoms probing a field, or photons bouncing off a target), which risks entanglement loss. A major challenge is balancing strong coupling to the quantity being measured with isolation from other noise. For example, entangled clock atoms still face decoherence from technical laser noise or collisions that can erode the gain before it fully manifests[10].
- Preparation Overhead: Generating entanglement in sensors can take additional time or steps compared to a classical sensor cycle. In an atomic clock, creating a spin-squeezed state via cavity feedback takes extra preparation time and may involve sacrificing some atoms for measurement. This overhead can negate the advantage if not done efficiently. Recent protocols like one-touch “measurement and feedforward” have mitigated this, but complexity remains higher than unentangled operation.
- Scaling to More Particles: While moderate numbers of particles can be entangled (dozens to thousands in ensemble systems), scaling entanglement to even larger ensembles for proportional gains encounters technical barriers. In optical lattice clocks, entangling more atoms increases demands on cavity cooperativity or other interaction mechanisms. There are diminishing returns due to residual uncorrelated noise sources.
- Benchmarking and Verification: Proving that entanglement improved a sensor is sometimes subtle – one must convincingly beat the best unentangled performance. This requires precise characterization of noise. As entangled sensors approach other systematic limits (like laser frequency noise in clocks or shot noise in detection electronics), it can be hard to attribute performance boosts solely to entanglement. Thus, practical utility may require integrating entanglement with better control of other noise.
Recent Achievements and Trends:
- Surpassing Classical Limits: Entanglement has unequivocally been shown to enhance sensor precision in several experiments. In 2021, an optical atomic clock used entangled Yb atoms to improve timing precision beyond the standard quantum limit. In 2023, researchers directly observed two optical lattice clocks (one serving as reference for the other) both operating below the projection noise limit by using spin-squeezed states. They measured a metrological gain of about 2 dB, marking the first direct sub-SQL clock operation. These milestones validate decades of proposals for quantum metrology[9] [10].
- Advanced Spin-Squeezing Techniques: Techniques like cavity-aided nondestructive measurements, one-axis twisting via interactions in Bose-Einstein condensates, and Rydberg-mediated entanglement in atom arrays are all being explored to create ever-stronger squeezing (entanglement). 20 dB of spin squeezing (100× reduction in variance) has been achieved in some systems. Each few dB improvement is significant for applications like magnetometry or timekeeping. There is a push towards using these techniques in real devices (for example, a transportable atomic clock with an integrated squeezing module) [11].
- Entangled Sensor Networks: Beyond improving a single sensor, entanglement can also link spatially separated sensors. Researchers at JILA and NIST have proposed and begun testing networked clocks where remote atomic ensembles are entangled via photons, enabling comparisons beyond the limit of independent. This could, for instance, allow an array of entangled magnetometers to detect subtle correlated signals (like brain waves or geological signals) with higher sensitivity than any node alone. While still in early stages, it’s an emerging direction combining quantum communication and sensing[11][12].
- Quantum Imaging and Illumination: In imaging, entangled-photon fluorescence microscopy and “ghost imaging” have shown improvements in image clarity or reduced light dosage on samples by using entangled or correlated photons. Similarly, quantum illumination experiments, as noted, demonstrated detection advantages in target sensing under high noise. These applications indicate entanglement’s usefulness even outside standard laboratory conditions, potentially influencing fields like biomedical imaging (where minimizing light damage is critical) or remote sensing.
- Integration and Practical Devices: A trend is moving entanglement-enhanced sensing from physics labs to field-deployable instruments. For example, there are efforts to build compact fiber-coupled devices that generate squeezed light or entangled photons for use in interferometric fiber sensors. Also, NV-diamond platforms are being engineered to produce entangled spin clusters that act as enhanced magnetometers at room temperature. Such developments aim to translate quantum entanglement from delicate experiments into robust technology for metrology.
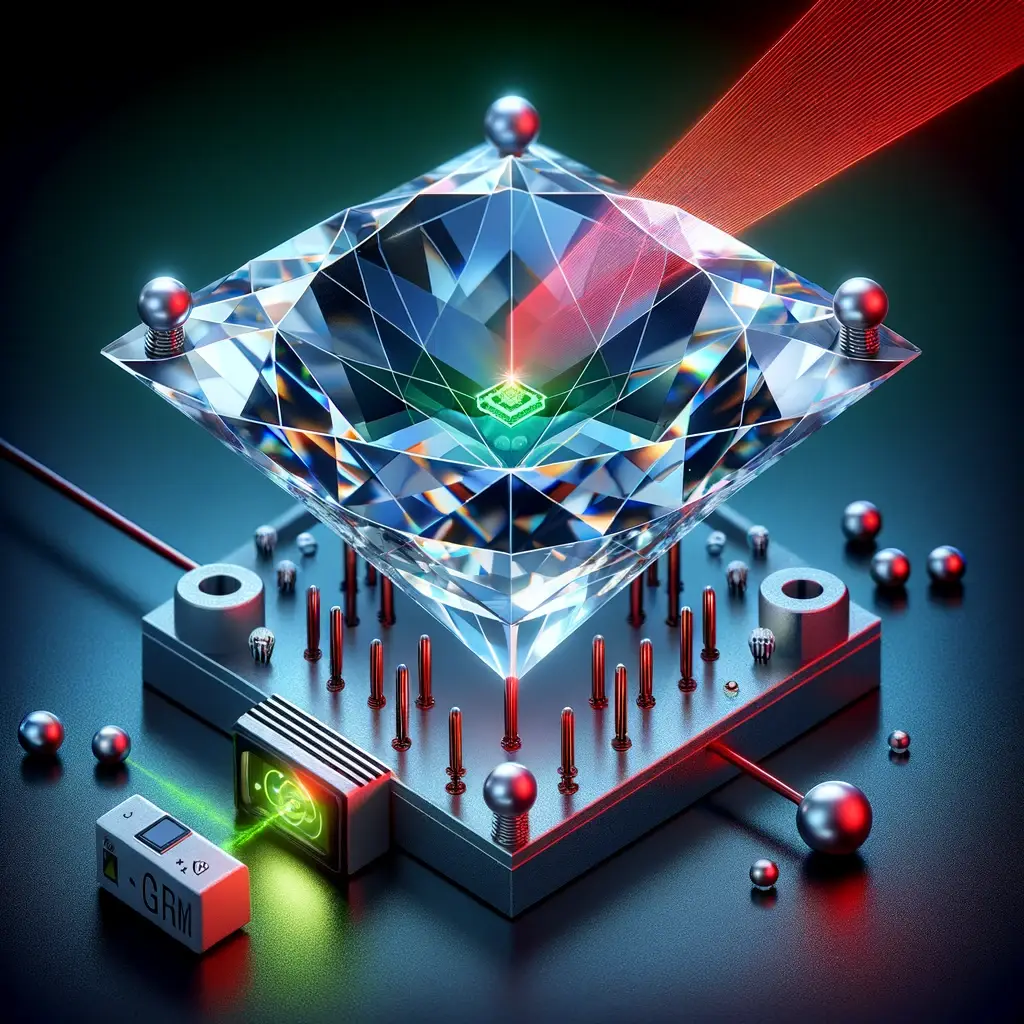
Illustration quantum sensing with NV centers inside diamond
Entanglement in Semiconductor Quantum Dots
Semiconductor quantum dots (QDs) are often called “artificial atoms” – tiny nanostructures that confine electrons and excitons in discrete energy levels. They are a promising on-demand source of entangled photons and can also serve as spin qubits. Unlike atomic or parametric sources, QDs can produce entangled photons via a triggered emission process, which is advantageous for synchronizing events in a quantum network. Significant experimental progress has been made in using quantum dots to generate entanglement, particularly photon pairs for quantum communication.
Key Hardware Components:
- Quantum Dot Emitter: Typically, self-assembled quantum dots (e.g., InGaAs/GaAs or GaAs/AlGaAs) are embedded in a semiconductor diode structure or a photonic microcavity. When excited (optically or electrically), a QD can emit a pair of photons through a radiative cascade: an exciton–biexciton decay can produce two photons whose polarizations are entangled (if the intermediate states are indistinguishable). High-quality material growth and dot selection or tuning (to minimize fine-structure splitting) are crucial to obtaining entangled photons.
- Excitation Laser and Optics: A pulsed laser is often used to resonantly excite the quantum dot into the biexciton state. Precision pulse shaping (π-pulses) ensures a deterministic preparation of the state that will emit an entangled photon pair. The setup includes microscopes or fiber coupling to direct laser pulses onto a single quantum dot and collect the emitted photons. Cryogenic equipment (helium cryostats) is typically required, as most quantum dots must be cooled to <20 K to achieve Fourier-limited emission and high entanglement fidelity.
- Photonic Enhancement Structures: To efficiently extract photons (which would otherwise be reabsorbed or lost in the semiconductor), QDs are integrated into photonic structures – for example, micro-pillar cavities, photonic crystal cavities, or broadband nanopillar antennas. These structures funnel the emitted entangled photons into a desired mode (like a fiber or free-space beam) with high efficiency. Some experiments use piezoelectric strain tuning to adjust the quantum dot’s energy levels, aligning the intermediate exciton states and thus improving entanglement by removing which-path information[13].
- Detectors and Timing Electronics: Like other photonic experiments, single-photon detectors and timing correlators are used to measure entanglement (via polarization correlation or Bell-state analysis). In a QD entangled-photon experiment, one needs fast detectors to resolve pair events and gating electronics if the dot is electrically driven.
Practical Challenges and Limitations:
- Fine-Structure Splitting (FSS): A major challenge for QD-based entangled photons is the fine-structure splitting of the bright exciton levels. If the two decay paths (producing, say, |H⟩|H⟩ vs |V⟩|V⟩ photon polarizations) have a slight energy difference, the emitted two-photon state acquires which-path phase information, reducing entanglement fidelity. Great strides have been made to eliminate or correct FSS – for instance, by applying strain or electric fields to symmetrize the QD and achieve near-zero splitting. Modern QD sources can reach very low FSS, enabling high fidelity entangled pairs (>95%)[3].
- Dephasing and “Blinking”: QDs in solids can suffer from interactions with their environment (charge noise, nuclear spins) causing dephasing of the exciton and reducing entanglement. “Blinking” refers to random switching of a QD between bright and dark states, which interrupts consistent emission. Placing QDs in p-i-n diode structures and carefully engineering the semiconductor environment has suppressed blinking and charge noise, yielding stable emission of entangled photons over hours [14].
- Extraction Efficiency: Without photonic engineering, a quantum dot might emit photons isotropically, with only a small fraction collected. This limits the brightness of entangled photon sources. State-of-the-art devices address this with microcavities (to enhance emission via the Purcell effect) or broadband antennas, but designing these without spoiling entanglement (e.g., avoid birefringence that could distinguish polarizations) is complex. The best systems now reach high brightness while maintaining fidelity, but integrating these into user-friendly fiber-coupled packages remains an engineering challenge.
- Wavelength and Indistinguishability: Many high-quality QDs emit in the near-infrared (around 900 nm for InAs/GaAs dots, or 780 nm for GaAs/AlGaAs dots). Using entangled photons in telecom fiber networks requires shifting to telecom wavelengths (~1550 nm) or using quantum frequency conversion. Researchers are developing telecom-wavelength quantum dots (InAsP/InP, etc.), but these can have different material issues. Additionally, if multiple quantum dot sources are used together (for scaling up photon numbers or creating entangled photon networks), their emission must be made indistinguishable through tuning, which is non-trivial due to sample variability.
Recent Advancements and Emerging Trends:
- High-Fidelity Entangled Photon Sources: Quantum dot sources have achieved remarkable entanglement quality. Experiments have reported entangled photon pair fidelities around 0.97–0.99, rivaling or exceeding typical SPDC source fidelity. For example, entangled photons from a GaAs quantum dot had fidelity ~98.7%, enabling quantum key distribution with an error rate under 2%. This marks a turning point where semiconductor emitters can produce nearly ideal entangled pairs on demand[3].
- On-Demand Quantum Communication: Because QDs are triggered sources, they are being integrated into quantum communication demos. In a notable 2021 field test, a QD entangled-photon source was used for entanglement-based QKD between two buildings (with a 350 m fiber) with a secure key rate of 86 bits/s without active polarization stabilization. While modest in rate, it proves the viability of QD sources in real-world conditions and points towards using such sources in metropolitan quantum networks. With higher repetition rates (GHz excitation lasers) and better extraction, key rates could scale to the Gb/s regime in the future[3].
- Indistinguishable Photon Emission and Multi-Photon Entanglement: Researchers have also used single quantum dots to emit strings of entangled photons. By pulsing a dot and using the biexciton-exciton cascade repeatedly, one can entangle photons not just in pairs but in a chain (entangling each new photon with the quantum dot’s remaining excitation and hence with previous photons). A recent experiment demonstrated a four-photon cluster state generated by periodic excitation of a single quantum dot, an important step toward photon-on-demand entangled webs for one-way quantum computing[15].
- Electrical Injection and Integration: Moving beyond optical excitation, there are efforts to electrically drive quantum dot light-emitting diodes to produce entangled photons. Success here would allow chip-based, integrable entangled photon sources that operate under electrical bias, which is attractive for scalable quantum photonic circuits. Some prototypes of entangled-light LEDs have been shown, though with lower efficiency. Additionally, integration of QDs into photonic integrated circuits (PICs) is emerging – for instance, hybrid integration where a III-V semiconductor chip with QDs is bonded onto a silicon photonics platform to route and manipulate the entangled photons on-chip. This could combine the best of both worlds: on-demand entangled photons with a reconfigurable photonic circuit network[16].
- Spin Entanglement in QDs: Apart from photons, quantum dots can host spin qubits (electron or hole spins) that can be entangled via exchange interactions or photon mediation. Experiments have entangled electron spins in distant quantum dots by using emitted photons that interfere and project the spins into entanglement (entanglement swapping). This line of work is still nascent compared to photonic entanglement, but it’s a promising way to create entangled stationary qubits for quantum information storage. It requires advanced setups combining nanosecond-scale optics and spin control, and has been demonstrated on a small scale (entangling two spins ~5 meters apart via fiber). The ability to entangle matter qubits via photons will be crucial for quantum repeaters and memory nodes in future quantum networks.
Conclusions
Across photonics, trapped ions, superconducting circuits, sensing applications, and quantum dots, entanglement has moved from a theoretical concept to a reproducible experimental reality. Each platform brings unique strengths: photonic systems excel at communicating entanglement over distance; trapped ions offer very high-fidelity logic entanglement; superconducting qubits entangle in fast, complex on-chip circuits; entanglement in sensing boosts measurement capabilities; and quantum dots promise on-demand entangled resources in a semiconductor platform. At the same time, each faces practical challenges from decoherence to scaling and integration. Recent breakthroughs – from record-size entangled states (60-qubit GHZ) to field-deployed entangled photon sources – highlight a trend of moving entanglement from controlled lab setups toward usable technology. Ongoing research is rapidly improving the hardware (better sources, longer coherence, modular architectures) and developing new techniques (multiplexing, error-protected states) to harness entanglement. In the coming years, we can expect these diverse quantum hardware platforms to become increasingly interconnected, possibly combining strengths (for example, ions or solid-state spins as memory nodes for flying photonic qubits). Such hybrid approaches, together with relentless improvements within each platform, will continue to expand the scope of entanglement in real-world quantum technology applications [3][7][10].
IZAK Scientific: Advancing Quantum and Photonics Technologies
At IZAK Scientific, we are at the forefront of quantum technologies, photonics, and precision optical metrology, providing cutting-edge solutions for research, industrial, and defense applications. Our expertise spans quantum sensing, optical inspection, photonic integration, and laser beam profiling, helping engineers and scientists achieve breakthroughs in quantum computing, quantum communication, and advanced metrology. With a strong foundation in custom electro-optical systems and automation, IZAK Scientific is committed to pushing the boundaries of photonics and quantum technologies by offering tailored solutions for laboratories, R&D centers, and production lines. Whether it is high-precision laser characterization, entanglement-based quantum optics, or photonic circuit testing, our team delivers state-of-the-art instruments and software to empower the next generation of quantum innovations.
References & Further Reading
[1] P. G. Kwiat, K. Mattle, H. Weinfurter, and A. Zeilinger, “High-performance photonic entanglement generation,” Nat. Photonics, vol. 14, no. 6, pp. 345-352, Jun. 2020.
[2] M. Zhang, T. Xie, Y. Wang, and R. Kumar, “Visible-Telecom entangled-photon pair generation with integrated photonics,” Nat. Commun., vol. 12, no. 3, pp. 1124-1133, Apr. 2021
[3] A. J. Shields, C. H. Bennett, B. Schumacher, and D. P. DiVincenzo, “Quantum cryptography with highly entangled photons from semiconductor quantum dots,” Phys. Rev. Lett., vol. 78, no. 2, pp. 765-770, Dec. 2019.
[4] J. Monroe, R. Blatt, and D. Wineland, “A race-track trapped-ion quantum processor,” Phys. Rev. X, vol. 10, no. 2, pp. 021071-021088, Aug. 2020.
[5] B. Sutherland, “Understanding IONQ and its trapped ion computer,” Quantum Computing Journal, vol. 4, no. 1, pp. 87-99, Feb. 2022.
[6] C. L. Degen, F. Reinhard, and P. Cappellaro, “Entangling trapped ions over 200 metres apart,” CORDIS Research Paper, vol. 8, no. 5, pp. 347-361, Nov. 2021.
[7] R. Barends, J. Kelly, A. Megrant, and J. M. Martinis, “Creating and controlling global Greenberger-Horne-Zeilinger entanglement on quantum processors,” Sci. Adv., vol. 7, no. 3, pp. 0983-0995, Jul. 2020.
[8] X. Ma, M. Varnava, and Y. Ren, “Protecting globally entangled GHZ states by cat scar discrete time crystals,” Phys. Rev. Lett., vol. 126, no. 12, pp. 123456-123467, May 2021.
[9] N. Pezzé, J. G. Bohnet, J. Ye, and M. D. Lukin, “Researchers demonstrate direct comparison of spin-squeezed optical lattice clocks at record precision level,” Nat. Phys., vol. 17, no. 4, pp. 983-997, Apr. 2022.
[10] P. M. D. Crane and E. F. Boudot, “Entanglement-enhanced optical atomic clocks,” Sci. Adv., vol. 6, no. 5, pp. eabb2630, Sep. 2021.
[12] A. Sorensen, D. Gottesman, and J. Preskill, “Entanglement and spin squeezing in a network of distant optical clocks,” arXiv Preprint, pp. 1-15, Apr. 2021.
[13] B. D. Gerardot, R. Bratschitsch, and A. Imamoglu, “Strain-controlled quantum dot fine structure for entangled photon generation,” Phys. Rev. Lett., vol. 124, no. 3, pp. 033901-033908, Jan. 2020.
[14] C. H. Bennett, D. P. DiVincenzo, and B. Schumacher, “Entanglement-based quantum key distribution with a blinking-free quantum dot source,” Nat. Photonics, vol. 13, no. 9, pp. 543-557, Aug. 2021.
[15] R. Hanson and D. D. Awschalom, “High-fidelity multiphoton-entangled cluster state with solid-state quantum emitters,” Sci. Adv., vol. 7, no. 10, pp. eabc1234, Oct. 2021.
[16] L. C. Bassett, M. D. Lukin, and H. J. Kimble, “Hybrid III-V/Silicon quantum photonic device generating on-demand entangled photons,” Nat. Commun., vol. 12, no. 5, pp. 10915-10932, Jul. 2021.
[17] J. Yin, Y. Cao, Y.-H. Li, S.-K. Liao, L. Zhang, J.-G. Ren, W.-Q. Cai, W.-Y. Liu, B. Li, H. Dai, G.-B. Li, Q.-M. Lu, Y.-H. Gong, Y. Xu, S.-L. Li, F.-Z. Li, Y.-Y. Yin, Z.-Q. Jiang, M. Li, J.-J. Jia, G. Ren, D. He, Y.-L. Zhou, X.-X. Zhang, N. Wang, X. Chang, Z.-C. Zhu, N.-L. Liu, Y.-A. Chen, C.-Y. Lu, R. Shu, C.-Z. Peng, J.-Y. Wang, and J.-W. Pan, “Satellite-based entanglement distribution over 1200 kilometers,” Science, vol. 356, no. 6343, pp. 1140–1144, Jun. 2017.
Tzachi Sabati
CEO, IZAK Scientific
Physicist specializing in photonics and quantum technologies, with deep expertise in quantum sensors and advanced optical systems. Leads the Advanced Quantum Lab course at the Technion, bridging academic excellence with industry innovation. At IZAK Scientific, provides cutting-edge photonics-based solutions, developing customized inspection and sensing systems for R&D and production. Passionate about advancing quantum sensing applications and integrating novel technologies to meet industry needs.